Programming Biomaterials
A conversation with Jessica about her work with silicone breast implants and hydrogels.
What do contact lenses, prosthetic heart valves, vaccines, and breast implants all have in common? They are all made out of biomaterials. A biomaterial is a substance that has been engineered to interact with biological systems. They can be derived from nature or synthesized in the lab using metallic components, polymers, ceramics, or composite materials.
Another thing that is in common with all of these things, they all make contact with our body and thus interact with our immune system. A super important question for all of these products is how can they evade (in the case of contact lenses, heart valves, and breast implants) or activate (in the case of vaccines) the immune system. These questions fall under the realm of immunology, a branch of biology and medicine that covers the study of immune systems in all organisms.
These two topics, immunology, and biomaterials, were the subject matter of my conversation with Jessica Stelzel, a Ph.D. student at Johns Hopkins University (JHU).
Background
Jessica attended Georgia Tech for her undergraduate studies with a passion for engineering, although she was unsure of which major to pursue. This is a common theme, as most people are usually uncertain about their exact path in college, and even those who think they know often change their minds. Jessica chose materials science because she became interested in how we can understand the properties of materials based on their structures.
One thing that Jessica was sure about was her desire to pursue a Ph.D. and remain in academia. This ambition brought her to Johns Hopkins, where she found the PIs to be particularly amazing. PI, or principal investigator, is a faculty member or research scientist appointed by the university to conduct research. At JHU, Jessica aimed to investigate how the immune system responds to biomaterials.
Jessica’s reasoning for pursuing academia was the autonomy it provides, allowing her to feel in control of her daily activities. Unlike industry research, which must be justified by profit or commercial potential, academia offers the freedom to explore any scientific question, provided you can secure funding through grants. This freedom enables more early-stage research, which may not be profitable now but could be crucial in the future.
More often than not, preclinical work happens in academia. To get a drug to market, several steps must be completed in both pre-clinical research and clinical trials:
Cell cultures: The first step, called in vitro research, involves testing your drug on cells in a petri dish, test tube, or labware.
Mice: If cell cultures prove successful, the next step is to test the drug in mice. Mice are used because they are genetically and biologically similar to humans, are small, easy to keep, and can be bred in large numbers.
Monkeys: Monkeys are also sometimes used because they are even more genetically similar to humans.
Humans: If everything else is successful, clinical trials on humans can begin, which are broken into four phases:
Phase I trials: Involves 20 to 100 healthy volunteers with the disease/condition, lasting several months. Approximately 70% of drugs move to the next phase.
Phase II trials: Includes up to several hundred people with the disease/condition, lasting several months to 2 years. Approximately 33% of drugs move to the next phase.
Phase III trials: Involves 300 to 3,000 people, lasting up to 4 years. Around 25%-30% of drugs move to the next phase.
Phase IV trials: Includes several thousand people, lasting several years.
Here is a great overview of all the steps to bring a drug to market (Source).
In the end, only one in ten drugs make it to patients, and the cost of bringing a drug to market is a ridiculous $1.3 billion. Clearly, there is a need for a more streamlined approach to identify failing drugs early, preventing wasted time and money. Moreover, there are significant differences between models, and optimizing a drug for one model doesn't guarantee it will work well in others, requiring repeated adjustments. What if we could replicate a human’s organs without actually using a human?
Introducing organ-on-a-chip, a multi-channel 3-D microfluidic chip that mimics the functions and responses of a whole organ or organ system. It’s not a functional organ but a combination of cell culture with microfluidics to emulate them. These models can simulate the human environment without the ethical concerns of using animals or humans, potentially allowing us to skip steps like mice and monkeys in the pre-clinical phase.
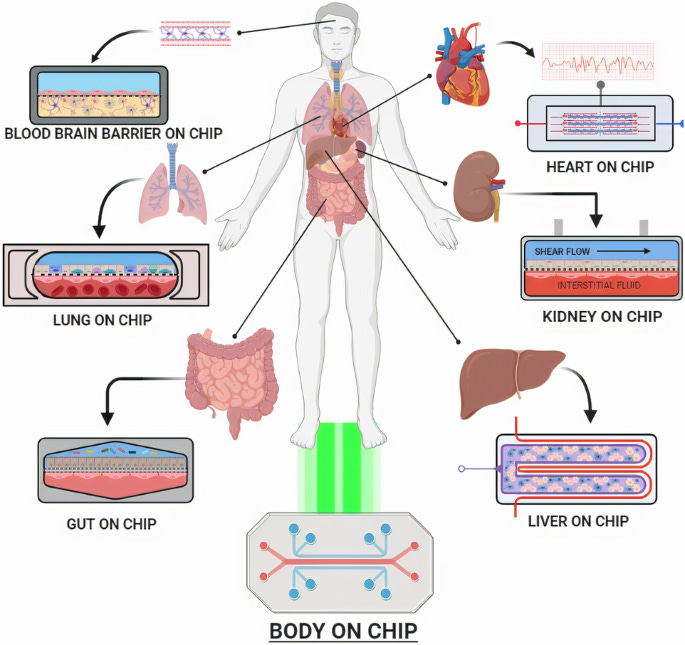
The FDA has already approved organ-on-a-chip technology. The Wyss Institute, a research and development institute at Harvard, already uses this technology to conduct research on a wide range of human diseases, including COVID-19, influenza, malnutrition, radiation exposure, and cystic fibrosis. However, organ-on-a-chip models are still new, and only recently have companies emerged in this space; Emulate is one of the leaders, actually providing organ-on-a-chip models for the Wyss Institute.
In addition to her research, Jessica co-taught a four-week undergraduate course at JHU focused on immunology and biomaterials. She taught the first half on immunology, while her labmate taught the part on biomaterials. The class explored questions such as the materials used for different medical applications, the meaning of biocompatibility, and the definition of the foreign body response. Jessica chose to develop and teach this course to see if she enjoyed teaching, as it is an option to pursue after her Ph.D; she in fact did enjoy this experience and it will definitely inform her future career decisions.
Jessica’s research - silicone breast implants
The last part of our conversation involved discussing Jessica’s research projects, both the ones she helped with and led herself. The first project focused on silicone breast implants and how the roughness of the implant’s surface influences the immune system’s foreign body response. This project was led by Jessica’s professor, Dr. Joshua Doloff.
Silicon, a material used in devices approved by the FDA, is generally considered biocompatible. Yet, the team found that even a material touted for its safety can elicit an immune response if the implant has a rough texture. Highly textured implants triggered a significant cytotoxic T cell response and even have been observed to lead to T cell-associated cancer (Breast Implant-Associated Anaplastic Large Cell Lymphoma (BIA-ALCL)). Cytotoxic T cells are a type of white blood cell that help the immune system fight germs and protect the body from disease.
Comparing textured implants with those having little texture (4 μm roughness being ideal), the team noticed more regulatory T cells (which calm cytotoxic T cells) around less textured implants. Conversely, highly textured implants lacked these calming regulatory T cells, resulting in a stronger immune response. Just by changing the texture, the immune response can be dramatically altered.
The lab studied the responses in mice and rabbits to both smooth and textured implants. Roughness was evaluated through techniques such as scanning electron microscopy and profilometry. Jessica specifically helped by using flow cytometry to confirm the presence or absence of fibroblasts. A major issue with these implants are processes called fibrosis and capsular contracture. In fibrosis, fibroblasts deposit collagen around the implants to wall them off. This is essentially the body’s way of saying that it doesn’t like whatever entered. Then, in capsular contracture, those collagen fibers shrink and squeeze the implant, which can cause medical complications. The team found that there were more activated fibroblasts in samples that had more surface texturing.
This research is incredibly significant beyond just breast implants, showing that even generally biocompatible materials can become unsafe and even carcinogenic (causing cancer) if certain properties, like surface texture, are altered. We need to be more cautious of generalizations as even materials that are generally considered biocompatible can be harmful in certain circumstances, as this study perfectly demonstrated.
Jessica’s research - nanofiber hydrogel composite
Nanofiber hydrogel composite is quite a mouthful so let’s break it down. The core component is the hydrogel, a jello-like polymer that can hold a lot of water and is chemically or physically crosslinked into a mesh. Hydrogels hold a lot of promise in the field of drug delivery, tissue engineering, and regenerative medicine because they are highly customizable and can be very easily injected with a needle. Hydrogels are customizable because of that crosslinking process, which we have only gotten better at doing as we become more familiar with the chemistry involved.
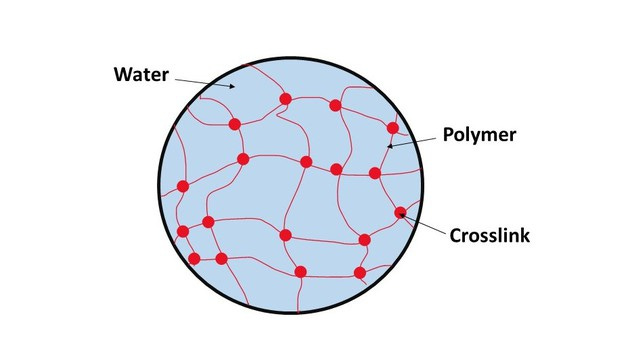
A nanofiber is a fiber, which is a material resembling a hair-like strand, on the nanoscale (a nanometer is a billionth of a meter). The composite means that you are combining two different types of materials, in this case the nanofiber with the hyaluronic acid, to create something new. Jessica’s composite was one that her lab already made, which involved crosslinking hyaluronic acid with a polycaprolactone (PCL) nanofiber.
Now I know I used the term crosslinking a lot without properly defining it. Crosslinking is the process of chemically joining two or more molecules by a covalent or ionic bond. Polymer chains on their own are sort of loose, but crosslinking intertwines those chains, making them more stable because they can’t move independently of each other. Think of a bunch of spaghetti, when it’s not intertwined, it is very loose and malleable; however, if you start attaching one piece of spaghetti to another, all of a sudden it can’t flow so easily and it is far more stiff and solid.
In the nanofiber hydrogel composite materials Jessica studies, the crosslinked hyaluronic acid hydrogel has additional covalent bonds with the PCL nanofibers because the nanofibers give the hydrogel a lot more stiffness while still maintaining large pores in the hydrogel. The size of the pores is an important parameter as pores are the holes in the crosslinked mesh that cells can pass through. To preface, Jessica is studying the hydrogel composite in the field of regenerative medicine, which involves using materials to replace or "regenerate" human cells, tissues, or organs to restore or establish normal function. Regenerative medicine involves creating materials that could act as a scaffold and cause immune infiltration into the material and then cause the material to be remodeled into something that looks like human tissue. Going back to why pores are so important, large pores are necessary for even cell distribution and connection throughout the engineered tissues and helps in the diffusion of nutrients and oxygen. These pores function somewhat similar to veins for a material that lacks a vascular system. Pore size also influences cell growth and tissue thickness, with there being optimal sizes for different types of tissue regeneration. For instance, skin regeneration requires pores of 20-125 μm, while bone regeneration needs 100-350 μm. Here is a good paper on this if you want to read more.
On a brief side tangent, a really exciting application of hydrogels is their use as a depot for drug delivery. A depot means that you can put the hydrogel under your skin and it can remain there for a while, slowly releasing the drug in the process. You can engineer the hydrogel through crosslinking to degrade easily or you can engineer them to be more solid and last longer. Often the drug is mixed in with the hydrogel, so as the hydrogel degrades so does the drug. However, the drug can also be directly attached to the hydrogel . Either way, the hydrogel degrades when enzymes travel through holes in the mesh and break down the crosslinks that connect the chains. Depending on how small you make the holes in the mesh, you can control where the cells and enzymes pass through and how quickly they do so. For example, you can make the holes on the inside of the hydrogel bigger so that it is easier for stuff to pass through the middle, meaning that the hydrogel will degrade from the inside. This amount of programmability with the hydrogel has made it so people have placed one drug on the inside and one in the outer layer, allowing for combinatorial therapy.
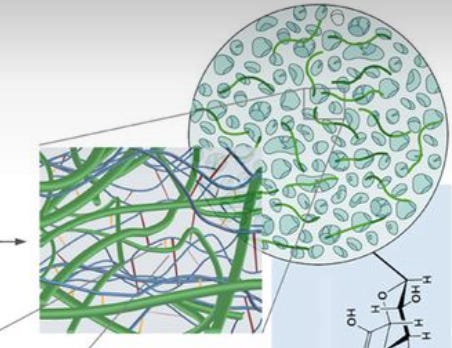
Jessica is tackling two essential questions surrounding this material: what immune cells are most pivotal towards causing tissue remodeling and what is the necessary amount of inflammation to produce wound healing properties? Jessica studied the microenvironment that the immune system created around it and initially noticed that there were inflammatory signals after the injection of the composite. This makes sense because the PCL fibers are known to be very inflammatory, which is super important because you can’t get tissue regeneration or remodeling without inflammation. While inflammation is often seen as a bad thing and something that we try to avoid, we have to remember that inflammation just means an immune response. If you don’t have inflammation, you won’t have any immune response and thus none of that wound healing process. Thus, inflammation should be seen as a positive thing in this context.
In order to find which immune cells are most pivotal towards tissue remodeling, Jessica knocked out, or in other words, eliminated immune cell types one at a time, waiting to see which immune cell when removed would cause the biggest decrease in remodeling.
The second question that Jessica tackled was inspired by the lab’s findings that when this composite was injected into animal tissue, it caused a bunch of blood vessel development, and the inside of the material got remodeled into soft adipose (fat) tissue. As a result, Jessica is trying to figure out the necessary amount of inflammation to get those wound healing properties: blood vessel development and the presence of soft adipose tissue. She is studying the cells that the material recruited and their function in this context. If she finds which cells are most vital for those wound healing properties, she can try to target the recruitment of only those cells, skewing the immune response towards those wound healing effects.
What is interesting about the hydrogel’s structure if you look back to that image is how it looks disconnected. This is because it was purposefully fragmented. The hydrogel was passed through a mesh screen so it can then be easily injected with a needle. Once it enters the body, the material forms back together into a cohesive structure due to the pressure in our body. This material and delivery method was developed with a Johns Hopkins plastic surgeon. He provides valuable input on whether their research would be adopted in the clinic and is a great example of the communication between the lab and the clinic. A big focus of the lab is how they can get their work translated, which is why they have a lot of clinical collaborators.
Conclusion
Wrapping up our conversation, Jessica mentioned her plans to become a professor after her amazing experience teaching a class. It really goes to show that you never know how much an experience can influence your trajectory. If you are presented with an opportunity to do something, always seize it. The worst that can happen is that you do the experience and find out that it was a waste of time; however, if you pass on an opportunity, who knows if that opportunity could have been a life-changing moment for you.
Jessica’s experience also definitely portrays the amazing parts of science research in academia. You are given far more freedom in the questions that you can explore and you are motivated by your passion for science rather than profit. It would be silly however to neglect the advantages of industry in that your research is far more likely to make contact with people. Academic research is largely focused on advancing the field of knowledge and doing cutting-edge research while industry research is often applying science to solve problems at the current moment. Regardless of where you go, having a strong understanding of the fundamentals and having those intangibles like problem-solving and resilience is absolutely essential. With those talents, you will succeed anywhere you go - industry, academia, or something totally separate from science.
Here are some additional sources besides that one that I already linked
https://www.fda.gov/patients/drug-development-process/step-3-clinical-research
https://en.wikipedia.org/wiki/Cost_of_drug_development#:~:text=A new study in 2020,drug development as %242.8 billion.